How is the Force like dark matter?
For Dark Matter Day, scientist and Star Wars fan Dan McKinsey talks dark matter and the Force.

Scientist Dan McKinsey of Berkeley Lab and UC Berkeley shares some thoughts on dark matter.
Ask Symmetry – How is the Force like dark matter?
McKinsey recently answered questions about dark matter on Reddit Science.
CERN alumna turned deep-sea explorer
Grace C. Young is fascinated by fundamental questions about realms both quantum and undersea.

Each summer, the international research laboratory CERN, home to the Large Hadron Collider, welcomes dozens of students to work alongside seasoned scientists on cutting-edge particle physics research. Many of these students will pursue physics research in graduate school, but some find themselves applying the lessons they learned at CERN to new domains.
In 2011, MIT undergraduate Grace Young was one of these CERN summer students.
Like many young adults, Young didn’t know what career path she wanted to pursue. “I tried all the majors,” Young says. “Physics, engineering, architecture, math, computer science. Separately, I always loved both the ocean and building things; it wasn’t until I learned about ocean engineering that I knew I had found my calling.”
Today, Young is completing her PhD in ocean engineering at the University of Oxford and is chief scientist for the deep-sea submarine Pisces VI. She develops technology for ocean research and in 2014 lived underwater for 15 days. During a recent visit to CERN, Young spoke with Symmetry writer Sarah Charley about the journey that led her from fundamental physics back to her first love, the ocean.
As a junior in high school you competed in Intel’s International Science Fair and won a trip to CERN. What was your project?
A classmate and I worked in a quantum physics lab at University of Maryland. We designed and built several devices, called particle traps, that had potential applications for quantum computing. We soldered wires onto the mirror inside a flashlight to create a bowl-shaped electric field and then applied alternating current to repeatedly flip the field, which made tiny charged particles hover in mid-air.
We were really jumping into the deep end on quantum physics; it was kind of amazing that it worked! Winning a trip to CERN was a dream come true. It was a transformative experience that had a huge impact on my career path.
You then came back to CERN as a freshman at MIT. What is it about CERN and particle physics that made you want to return?
My peek inside CERN the previous year sparked an interest that drove me to apply for the Openlab internship [a technology development collaboration between CERN scientists and members of companies or research institutes].
Although I learned a lot from my assignment, my interest and affinity for CERN derives from the community of researchers from diverse backgrounds and disciplines from all over the world. It was CERN's high-powered global community of scientists congregated in one beautiful place to solve big problems that was a magnet for me.
You say you’ve always loved the ocean. What is it about the ocean that inspires you?
I’ve loved being by the water since I was born. I find it very humbling, standing on the shore and having the waves breaking at my feet.
This huge body of water differentiates our planet from other rocks in space, yet so little is known about it. The more time I spent on or in the water, either sailing or diving, the more I began taking a deeper interest in marine life and the essential role the ocean plays in sustaining life as we know it on Earth.
What does an ocean engineer actually do?
One big reason that we’ve only explored 5 percent of the ocean is because the deep sea is so forbidding for humans. We simply don't have the biology to see or communicate underwater, much less exist for more than a few minutes just below surface.
But all this is changing with better underwater imaging, sensors and robotic technologies. As an ocean engineer, I design and build things such as robotic submersibles, which can monitor the health of fisheries in marine sanctuaries, track endangered species and create 3-D maps of underwater ice shelves. These tools, combined with data collected during field research, enable me and my colleagues to explore the ocean and monitor the human impact on its fragile ecosystems.
I also design new eco-seawalls and artificial coral reefs to protect coastlines from rising sea levels and storm surges while reviving essential marine ecosystems.
What questions are you hoping to answer during your career as an ocean engineer and researcher?
How does the ocean support so much biodiversity? More than 70 percent of our planet is covered by water, producing more than half the oxygen we breathe, storing more carbon dioxide than all terrestrial plant life and feeding billions of humans. And yet 95 percent of our ocean remains unexplored and essentially unknown.
The problem we are facing today is that we are destroying so many of the ocean’s ecosystems before we even know they exist. We can learn a lot about how to stay alive and thrive by studying the oceanic habitats, leading to unforeseeable discoveries and scientific advancements.
What are some of your big goals with this work?
We face big existential ocean-related problems, and I'd like to help develop solutions for them. Overfishing, acidification, pollution and warming temperatures are destroying the ocean’s ecosystems and affecting humans by diminishing a vital food supply, shifting weather patterns and accelerating sea-level rise. Quite simply, if we don't know or understand the problems, we can't fix them.
Have you found any unexpected overlaps between the research at CERN and the research on a submarine?
Vision isn’t a good way to see the underwater world. The ocean is pitch black in most of its volume, and the creatures don’t rely on vision. They feel currents with their skin, use sound and can read the chemicals in the water to smell food. It would make sense for humans to use sensors that do that same thing.
Physicists faced this same challenge and found other ways to characterize subatomic particles and the celestial bodies without relying on vision. Ocean sciences are moving in this same direction.
What do you think ocean researchers and particle physicists can learn from each other?
I think we already know it: That is, we can only solve big problems by working together. I'm convinced that only by working together across disciplines, ethnicities and nationalities can we survive as a species.
Of course, the physical sciences are integral to everything related to ocean engineering, but it's really CERN's problem-solving methodology that's most inspiring and applicable. CERN was created to solve big problems by combining the best of human learning irrespective of nationality, ethnicity or discipline. Our Pisces VI deep sea submarine team is multidisciplinary, multinational and—just like CERN—it's focused on exploring the unknown that's essential to life as we know it.
Speak physics: What is a cross section?
Cross sections tell physicists how likely particles are to interact in a given way.

Imagine two billiard balls rolling toward one another. The likelihood of a collision depends on easy-to-grasp concepts: How big are they? How precisely are they aimed?
When you start talking about the likelihood of particles colliding, things get trickier. That’s why physicists use the term “cross section.”
Unlike solid objects, elementary particles themselves behave as tiny waves of probability.
And their interactions are not limited to a physical bump. Particles can interact at a distance, for example, through the electromagnetic force or gravity. Some particles, such as neutrinos, interact only rarely through the weak force. You might imagine them as holograms of billiard balls that occasionally flit into a solid state.

In physics, a cross section describes the likelihood of two particles interacting under certain conditions. Those conditions include, for example, the number of particles in the beam, the angle at which they hit the target, and what the target is made of.
“Cross sections link theory with reality,” says Gerardo Herrera, a researcher at the Center for Research and Advanced Studies of the National Polytechnic Institute in Mexico City and a collaborator on the ALICE experiment at the Large Hadron Collider. “They provide a picture of the fundamental properties of particles. That’s their greatest utility.”
Cross sections come in many varieties. They can help describe what happens when a particle hits a nucleus. In elastic reactions, particles bounce off one another but maintain their identities, like two ricocheting billiard balls. In inelastic reactions, one or more particle shatters apart, like a billiard ball struck by a bullet. In a resonance state, short-lived virtual particles appear.

These measurements of one or more aspects of the interaction are called differential cross sections, while summaries of all of these reactions put together are called total cross sections.
Physicists represent cross sections in equations with the Greek letter sigma (σ). But once they have been measured in actual collisions, their data can be visualized in figures like this:
This plot comes from a paper on interactions between neutrinos and atomic nuclei. The vertical axis represents the chances of the different reactions (measured in square centimeters over giga-electronvolts), and the horizontal axis represents the energy of the incoming neutrinos (measured in giga-electronvolts). An electronvolt is a measure of energy based on the amount of energy an electron gains after being accelerated by 1 volt of electricity.
The above image is telling us, for instance, that at an energy of 10 giga-electronvolts the most probable result would be a deep inelastic scattering (green line), followed by a resonance state (red line), and lastly by a quasi-elastic event (blue line). The black curve represents the total cross section. The error bars (thin lines that go sideways and upside-down) indicate the estimated accuracy of each measurement.
“What you see in this figure are attempts to find a common way to display complex experimental results. This plot is showing how we divide up events that we find in our detectors,” says Jorge Morfín, a senior scientist at Fermilab and one of the main authors of the paper.
Cross sections are used to communicate results among researchers with common interests, Morfín says. The previous cross section serves, then, as a way to compare data obtained from labs that use different measurement techniques and nuclear targets, such as NOMAD (CERN), SciBooNE (Fermilab) and T2K (Japan).
Scientists studying astrophysics, quantum chromodynamics, physical chemistry and even nanoscience use these kinds of plots in order to understand how particles decay, absorb energy and interact with one another.

“They make so many connections with different scientific fields and current research that’s going on,” says Tom Abel, a computational cosmologist at SLAC National Accelerator Laboratory and Stanford University.
In the hunt for dark matter, for example, researchers investigate whether particles interact in the way theorists predict.
“We are looking for interactions between dark matter particles and heavy nuclei, or dark matter particles interacting with one another,” Abel says. “All of this is expressed in cross-sections.”
If they see different interactions than they expect, it could be a sign of the influence of something unseen—like dark matter.
In a world where probability and uncertainty reign, Herrera notes that concepts in quantum mechanics can be difficult to grasp. “But cross sections are a very tangible element,” he says, “and one of the most important measurements in high-energy physics.”
Scientists make rare achievement in study of antimatter
Through hard work, ingenuity and a little cooperation from nature, scientists on the BASE experiment vastly improved their measurement of a property of protons and antiprotons.

Scientists at CERN are celebrating a recent, rare achievement in precision physics: Collaborators on the BASE experiment measured a property of antimatter 350 times as precisely as it had ever been measured before.
The BASE experiment looks for undiscovered differences between protons and their antimatter counterparts, antiprotons. The result, published in the journal Nature, uncovered no such difference, but BASE scientists say they are hopeful the leap in the effectiveness of their measurement has potentially brought them closer to a discovery.
“According to our understanding of the Standard Model [of particle physics], the Big Bang should have created exactly the same amount of matter and antimatter, but [for the most part] only matter remains,” says BASE Spokesperson Stefan Ulmer. This is strange because when matter and antimatter meet, they annihilate one another. Scientists want to know how matter came to dominate our universe.
“One strategy to try to get hints to understand the mechanisms behind this matter-antimatter symmetry is to compare the fundamental properties of matter and antimatter particles with ultra-high precision,” Ulmer says.
Scientists on the BASE experiment study a property called the magnetic moment. The magnetic moment is an intrinsic value of particles such as protons and antiprotons that determines how they will orient in a magnetic field, like a compass. Protons and antiprotons should behave exactly the same, other than their charge and direction of orientation; any differences in how they respond to the laws of physics could help explain why our universe is made mostly of matter.
This is a challenging measurement to make with a proton. Measuring the magnetic moment of an antiproton is an even bigger task. To prevent antiprotons from coming into contact with matter and annihilating, scientists need to house them in special electromagnetic traps.
While antiprotons generally last less than a second, the ones used in this study were placed in a unique reservoir trap in 2015 and used one by one, as needed, for experiments. The trapped antimatter survived for more than 400 days.
During the last year, Ulmer and his team worked to improve the precision of the most sophisticated technqiues developed for this measurement in the last decade.
They did this by improving thier cooling methods. Antiprotons at temperatures close to absolute zero move less than room-temperature ones, making them easier to measure.
Previously, BASE scientists had cooled each individual antiproton before measuring it and moving on to the next. With the improved trap, the antiprotons stayed cool long enough for the scientists to swap an antiproton for a new one as soon as it became too hot.
“Developing an instrument stable enough to keep the antiproton close to absolute zero for 4-5 days was the major goal,” says Christian Smorra, the first author of the study.
This allowed them to collect data more rapidly than ever before. Combining this instrument with a new technique that measures two particles simultaneously allowed them to break their own record from last year’s measurement by a longshot.
“This is very rare in precision physics, where experimental efforts report on factors of greater than 100 magnitude in improvement,” Ulmer says.
The results confirm that the two particles behave exactly the same, as the laws of physics would predict. So the mystery of the imbalance between matter and antimatter remains.
Ulmer says that the group will continue to improve the precision of their work. He says that, in five to 10 years, they should be able to make a measurement at least twice as precise as this latest one. It could be within this range that they will be able to detect subtle differences between protons and antiprotons.
“Antimatter is a very unique probe,” Ulmer says. “It kind of watches the universe through very different glasses than any matter experiments. With antimatter research, we may be the only ones to uncover physics treasures that would help explain why we don’t have antimatter anymore.”
Scientists observe first verified neutron-star collision
For the first time, experiments have seen both light and gravitational waves released by a single celestial crash.

Today scientists announced the first verified observation of a neutron star collision. LIGO detected gravitational waves radiating from two neutron stars as they circled and merged, triggering 50 additional observational groups to jump into action and find the glimmer of this ancient explosion.
This observation represents the first time experiments have seen both light and gravitational waves from a single celestial crash, unlocking a new era of multi-messenger astronomy.
On August 17 at 7:41 am Eastern Time, NASA astronomer Julie McEnery had just returned from an early morning row on the Anacostia River when her experiment, the Fermi Gamma Ray Space Telescope, sent out an automatic alert that it had just recorded a burst of gamma rays coming from the southern constellation Hydra. By itself, this wasn’t novel; the Gamma-ray Burst Monitor instrument on Fermi has seen approximately 2 gamma-ray outbursts per day since its launch in 2008.
“Forty minutes later, I got an email from a colleague at LIGO saying that our trigger has a friend and that we should buckle up,” McEnery says.
Most astronomy experiments, including the Fermi Gamma Ray Space Telescope, watch for light or other particles emanating from distant stars and galaxies. The LIGO experiment, on the other hand, listens for gravitational waves. Gravitational waves are the equivalent of cosmic tremors, but instead of rippling through layers of rock and dirt, they stretch and compress space-time itself.
Exactly 1.7 seconds before Fermi noticed the gamma ray burst, a set of extremely loud gravitational waves had shaken LIGO’s dual detectors.
“The sky positions overlapped, strongly suggesting the two signals were coming from the same astronomical event,” says Daniel Holz, a professor at the University of Chicago and member of LIGO collaboration and the Dark Energy Survey Gravitational Wave group.
LIGO reconstructed the location and distance of the event and sent an alert to their allied astronomers. About 12 hours later, right after sunset, multiple astronomical surveys found a glowing blue dot just above the horizon in the area LIGO predicted.
“It lasted for two weeks, and we observed it for about an hour every night,” says Jim Annis, a researcher at the US Department of Energy’s Fermi National Accelerator Laboratory, the lead institution on the Dark Energy Survey. “We used telescopes that could see everything from low-energy radio waves all the way to high-energy X-rays, giving us a detailed image of what happened immediately after the initial collision.”
Neutron stars are roughly the size of the island of Nantucket but have more mass than the sun. They have such a strong gravitational pull that all their matter has been squeezed and transformed into a single, giant atomic nucleus consisting entirely of neutrons.
“Right before two neutron stars collide, they circle each other about 100 times a second,” Annis says. “As they collide, huge electromagnetic tornados erupt at the poles and material is sprayed out in all directions at close to the speed of light.”
As they merge, neutron stars release a quick burst of gamma radiation and then a spray of decompressing neutron star matter. Exotic heavy elements form and decay, dumping enough energy that the surface reaches temperatures of 20,000 degrees Kelvin. That's almost four times hotter than the surface of the sun and much brighter. Scientists theorize that a good portion of the heavy elements in our universe, such as gold, originated in neutron star collisions and other massively energetic events.
Since coming online in September 2015, the US-based LIGO collaboration and their Italy-based partners, the Virgo collaboration, have reported detecting five bursts of gravitational waves. Up until now, each of these observations has come from a collision of black holes.
“When two black holes collide, they emit gravitational waves but no light,” Holz says. “But this event released an enormous amount of light and numerous astronomical surveys saw it. Hearing and seeing the event provides a goldmine of information, and we will be mining the data for years to come.”
This is a Rosetta Stone-type discovery, Holz says. “We’ve learned about the processes that neutron stars are undergoing as they fling out matter and how this matter synthesizes into some of the elements we find on Earth, such as gold and platinum,” he says. “In addition to teaching us about mysterious gamma-ray bursts, we can use this event to calculate the expansion rate of the universe. We will be able to estimate the age and composition of the universe in an entirely new way.”
For McEnery, the discovery ushers in a new age of cooperation between gravitational-wave experiments and experiments like her own.
“The light and gravitational waves from this collision raced each other across the cosmos for 130 million years and hit earth 1.7 seconds apart,” she says. “This shows that both are moving at the speed of light, as predicted by Einstein. This is what we’ve been hoping to see.”
Xenon takes a turn in the LHC
For the first time, the Large Hadron Collider is accelerating xenon nuclei for experiments.

Most of the year, the Large Hadron Collider at CERN collides protons. LHC scientists have also accelerated lead nuclei stripped of their electrons. Today, for just about eight hours, they are experimenting with a different kind of nucleus: xenon.
Xenon is a heavy noble gas that exists in trace quantities in the air. Xenon nuclei are about 40 percent lighter than lead nuclei, so xenon-xenon collisions have a different geometry and energy distribution than lead-lead collisions.
“When two high-energy nuclei collide, they can momentarily form a droplet of quark gluon plasma, the primordial matter that filled our universe just after the big bang,” says Peter Steinberg, a physicist at the US Department of Energy’s Brookhaven National Laboratory and a heavy-ion coordinator for the ATLAS experiment at CERN. “The shape of the colliding nuclei influences the initial shape of this droplet, which in turn influences how the plasma flows and finally shows up in the angles of the particles we measure. We’re hoping that these smaller droplets from xenon-xenon collisions give us deeper insight into how this still-mysterious process works at truly subatomic length scales.”
Not all particles that travel through CERN’s long chain of interconnected accelerators wind up in the LHC. Earlier this year, scientists were loading xenon ions into the accelerator and firing them at a fixed-target experiment instead.
“We can have particles from two different sources feeding into CERN’s accelerator complex,” says Michaela Schaumann, a physicist in LHC operation working on the heavy-ion program. “The LHC’s injectors are so flexible that, once everything is set up properly, they can alternate between accelerating protons and accelerating ions a few times a minute.”
Having the xenon beam already available provided an opportunity to send xenon into the LHC for first (and potentially only) time. It took some serious additional work to bring the beam quality up to collider levels, Schaumann says, but today it was ready to go.
“We are keeping the intensities very low in order to fulfil machine protection requirements and be able to use the same accelerator configuration we apply during the proton-proton runs with xenon beams,” Schaumann says. “We needed to adjust the frequency of the accelerator cavities [because more massive xenon ions circulate more slowly than protons], but many of the other machine settings stayed roughly the same.”
This novel run tests scientists’ knowledge of beam physics and shows the flexibility of the LHC. Scientists say they are hopeful it could reveal something new.
“We can learn a lot about the properties of the hot, dense matter from smaller collision systems,” Steinberg says. “They are a valuable bridge to connect what we observe in lead-lead collisions to strikingly similar observations in proton-proton interactions.”
A play in parallel universes
Constellations illustrates the many-worlds interpretation of quantum mechanics—with a love story.

The play Constellations begins with two people, Roland and Marianne, meeting for the first time. It’s a short scene, and it doesn’t go well. Then the lights go down, come back up, and it’s as if the scene has reset itself. The characters meet for the first time, again, but with slightly different (still unfortunate) results.
The entire play progresses this way, showing multiple versions of different scenes between Roland, a beekeeper, and Marianne, an astrophysicist.
In the script, each scene is divided from the next by an indented line. As the stage notes explain: “An indented rule indicates a change in universe.”
To scientist Richard Partridge, who recently served as a consultant for a production of Constellations at TheatreWorks Silicon Valley, it’s a play about quantum mechanics.
“Quantum mechanics is about everything happening at once,” he says.
We don’t experience our lives this way, but atoms and particles do.
In 1927, physicists Niels Bohr and Werner Heisenberg wrote that, on the scale of atoms and smaller, the properties of physical systems remain undefined until they are measured. Light, for example, can behave as a particle or a wave. But until someone observes it to be one or the other, it exists in a state of quantum superposition: It is both a particle and a wave at the same time. When a scientist takes a measurement, the two possibilities collapse into a single truth.
Physicist Erwin Schrodinger illustrated this with a cat. He created a thought experiment in which the decay of an atom—an event ruled by quantum mechanics—would trigger toxic gas to be released in a steel chamber with a cat inside. By the rules of quantum mechanics, until someone opened the chamber, the cat existed in a state of superposition: simultaneously alive and dead.
Some interpretations of quantum mechanics dispute the idea that observing a system can determine its true state. In the many-worlds interpretation, every possibility exists in a giant collection of parallel realities. In some, the cat lives. In others, it does not.
In some Constellations universes, the astrophysicist and the beekeeper fall in love. In others, they do not. “So it’s not really about physics,” Partridge says.
Constellations director Robert Kelley, who founded TheatreWorks in 1970, agrees. He says he was intimidated by the physics concepts in the play at first but that he was eventually drawn to the relationship at its core.
“With all of these things swirling around in the play, what really counts is the relationship between two people and the love that grows between them,” he says. “I found that a very charming message for Silicon Valley. We’re surrounded by a whole lot of technology, but probably for most people what counts is when you get home and you’re on the couch and your one-and-a-half-year-old shows up.”
TheatreWorks in Silicon Valley production of Constellations

Cosmologist Marianne (Carie Kawa) and beekeeper Roland (Robert Gilbert) explore the ever-changing mystery of “what ifs” in the regional premiere of Constellations presented by TheatreWorks Silicon Valley, August 23-September 17, at the Mountain View Center for the Performing Arts.

Cosmologist Marianne (Carie Kawa) and beekeeper Roland (Robert Gilbert) explore the ever-changing mystery of “what ifs” in the regional premiere of Constellations presented by TheatreWorks Silicon Valley, August 23-September 17, at the Mountain View Center for the Performing Arts.

Cosmologist Marianne (Carie Kawa) and beekeeper Roland (Robert Gilbert) explore the ever-changing mystery of “what ifs” in the regional premiere of Constellations presented by TheatreWorks Silicon Valley, August 23-September 17, at the Mountain View Center for the Performing Arts.

Cosmologist Marianne (Carie Kawa) and beekeeper Roland (Robert Gilbert) explore the ever-changing mystery of “what ifs” in the regional premiere of Constellations presented by TheatreWorks Silicon Valley, August 23-September 17, at the Mountain View Center for the Performing Arts.

Cosmologist Marianne (Carie Kawa) and beekeeper Roland (Robert Gilbert) explore the ever-changing mystery of “what ifs” in the regional premiere of Constellations presented by TheatreWorks Silicon Valley, August 23-September 17, at the Mountain View Center for the Performing Arts.

Cosmologist Marianne (Carie Kawa) and beekeeper Roland (Robert Gilbert) explore the ever-changing mystery of “what ifs” in the regional premiere of Constellations presented by TheatreWorks Silicon Valley, August 23-September 17, at the Mountain View Center for the Performing Arts.

Cosmologist Marianne (Carie Kawa) and beekeeper Roland (Robert Gilbert) explore the ever-changing mystery of “what ifs” in the regional premiere of Constellations presented by TheatreWorks Silicon Valley, August 23-September 17, at the Mountain View Center for the Performing Arts.
Kelley says that he found something familiar in the many timelines of the play. “It’s really kind of fun to see all that happen because it’s common ground for us as human beings: You hang up the phone and think, ‘If only I’d said that or hadn’t said that.’ It’s a fascinating thought that every single thing that happens will then determine every single other thing that happens.”
Constantly resetting and replaying the same scenes “was very acrobatic,” says Los Angeles-based actress Carie Kawa, who played Marianne in the TheatreWorks production, which concluded in September. “And there were emotional acrobatics—just jumping into different emotional states. Usually you get a little longer arc; this play is just all middles, almost like shooting a film.”
To her, the repeats and jumps were familiar in a different way: They were an encapsulation of the experience of acting.
“We do the play over and over again,” she says. “It’s the same scene, but it’s different every single time. And if we’re doing it right, we’re not thinking about the scene that just happened or the scene that’s to come, we’re in the moment.”
The play will mean different things to different people, Kawa says.
“A teacher once told me a story about theater and a perspective that he had,” she says. “At first he said, ‘Theater is important because everybody can come together and feel the same feeling at the same time and know that we’re all okay.’
“But as he progressed in this artistry he realized that, no, what’s happening is everybody is feeling a slightly different feeling at the same time. And that’s OK. That’s what helps us experience our humanity and the humanity of the other people around us. We’re all alone in this together.”
A radio for dark matter
Instead of searching for dark matter particles, a new device will search for dark matter waves.

Researchers are testing a prototype “radio” that could let them listen to the tune of mysterious dark matter particles.
Dark matter is an invisible substance thought to be five times more prevalent in the universe than regular matter. According to theory, billions of dark matter particles pass through the Earth each second. We don’t notice them because they interact with regular matter only very weakly, through gravity.
So far, researchers have mostly been looking for dark matter particles. But with the dark matter radio, they want to look for dark matter waves.
Direct detection experiments for dark matter particles use large underground detectors. Researchers hope to see signals from dark matter particles colliding with the detector material. However, this only works if dark matter particles are heavy enough to deposit a detectable amount energy in the collision.
“If dark matter particles were very light, we might have a better chance of detecting them as waves rather than particles,” says Peter Graham, a theoretical physicist at the Kavli Institute for Particle Astrophysics and Cosmology, a joint institute of Stanford University and the Department of Energy’s SLAC National Accelerator Laboratory. “Our device will take the search in that direction.”
The dark matter radio makes use of a bizarre concept of quantum mechanics known as wave-particle duality: Every particle can also behave like a wave.
Take, for example, the photon: the massless fundamental particle that carries the electromagnetic force. Streams of them make up electromagnetic radiation, or light, which we typically describe as waves—including radio waves.
The dark matter radio will search for dark matter waves associated with two particular dark matter candidates. It could find hidden photons—hypothetical cousins of photons with a small mass. Or it could find axions, which scientists think can be produced out of light and transform back into it in the presence of a magnetic field.
“The search for hidden photons will be completely unexplored territory,” says Saptarshi Chaudhuri, a Stanford graduate student on the project. “As for axions, the dark matter radio will close gaps in the searches of existing experiments.”
Intercepting dark matter vibes
A regular radio intercepts radio waves with an antenna and converts them into sound. What sound depends on the station. A listener chooses a station by adjusting an electric circuit, in which electricity can oscillate with a certain resonant frequency. If the circuit’s resonant frequency matches the station’s frequency, the radio is tuned in and the listener can hear the broadcast.
The dark matter radio works the same way. At its heart is an electric circuit with an adjustable resonant frequency. If the device were tuned to a frequency that matched the frequency of a dark matter particle wave, the circuit would resonate. Scientists could measure the frequency of the resonance, which would reveal the mass of the dark matter particle.
The idea is to do a frequency sweep by slowly moving through the different frequencies, as if tuning a radio from one end of the dial to the other.
The electric signal from dark matter waves is expected to be very weak. Therefore, Graham has partnered with a team led by another KIPAC researcher, Kent Irwin. Irwin’s group is developing highly sensitive magnetometers known as superconducting quantum interference devices, or SQUIDs, which they’ll pair with extremely low-noise amplifiers to hunt for potential signals.
In its final design, the dark matter radio will search for particles in a mass range of trillionths to millionths of an electronvolt. (One electronvolt is about a billionth of the mass of a proton.) This is somewhat problematic because this range includes kilohertz to gigahertz frequencies—frequencies used for over-the-air broadcasting.
“Shielding the radio from unwanted radiation is very important and also quite challenging,” Irwin says. “In fact, we would need a several-yards-thick layer of copper to do so. Fortunately we can achieve the same effect with a thin layer of superconducting metal.”
One advantage of the dark matter radio is that it does not need to be shielded from cosmic rays. Whereas direct detection searches for dark matter particles must operate deep underground to block out particles falling from space, the dark matter radio can operate in a university basement.
The researchers are now testing a small-scale prototype at Stanford that will scan a relatively narrow frequency range. They plan on eventually operating two independent, full-size instruments at Stanford and SLAC.
“This is exciting new science,” says Arran Phipps, a KIPAC postdoc on the project. “It’s great that we get to try out a new detection concept with a device that is relatively low-budget and low-risk.”
The dark matter disc jockeys are taking the first steps now and plan to conduct their dark matter searches over the next few years. Stay tuned for future results.

The dark matter radio disc jockeys. Front row, from left: Carl Dawson, Hsiao-Mei “Sherry” Cho and Saptarshi Chaudhuri. Back row, from left: Arran Phipps, Stephen Kuenstner and Kent Irwin. Not pictured: Dale Li and Peter Graham.
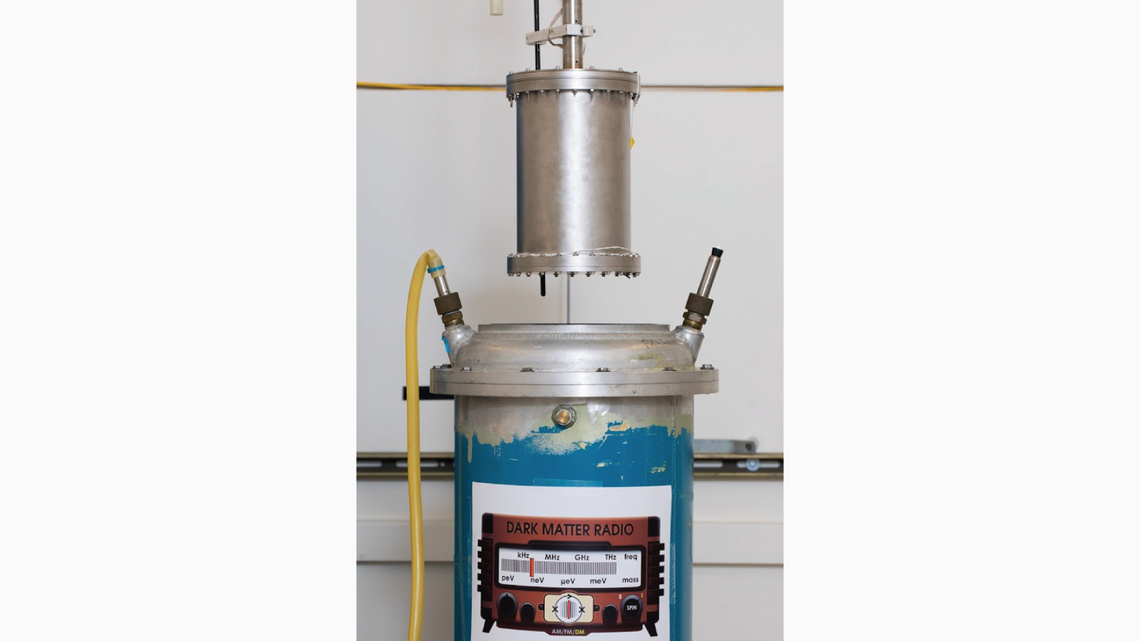
The dark matter radio is placed inside a 2-millimeter-thick superconducting niobium shield. When lowered into the blue dewar and cooled by liquid helium, the shield blocks interference from outside sources, such as radio stations, but is easily penetrated by dark matter.
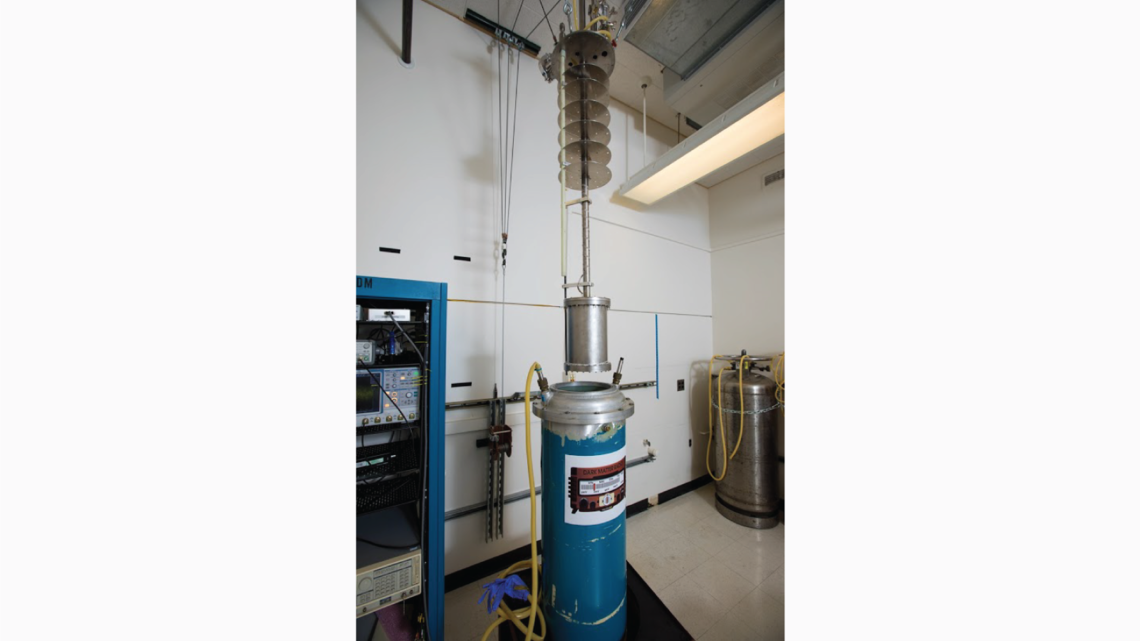
Layers of thermal barriers (top) are used to block heat from the laboratory and keep the radio cold. A cable inside the center rod brings signals from inside the shield to the outside world.

Dark matter waves should oscillate at a specific frequency corresponding to the mass of the dark matter particle. A spike in power will occur if the radio is tuned to match that frequency.

It takes about one day to cool the probe from room temperature to the operating temperature of negative 450 degrees Fahrenheit.